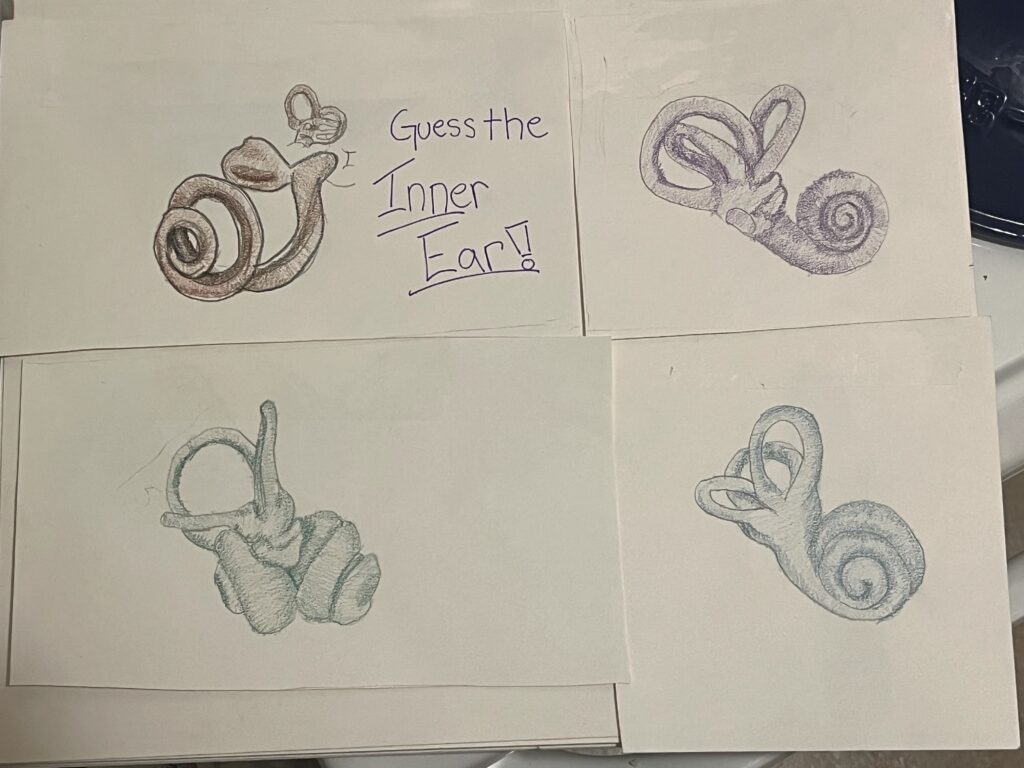
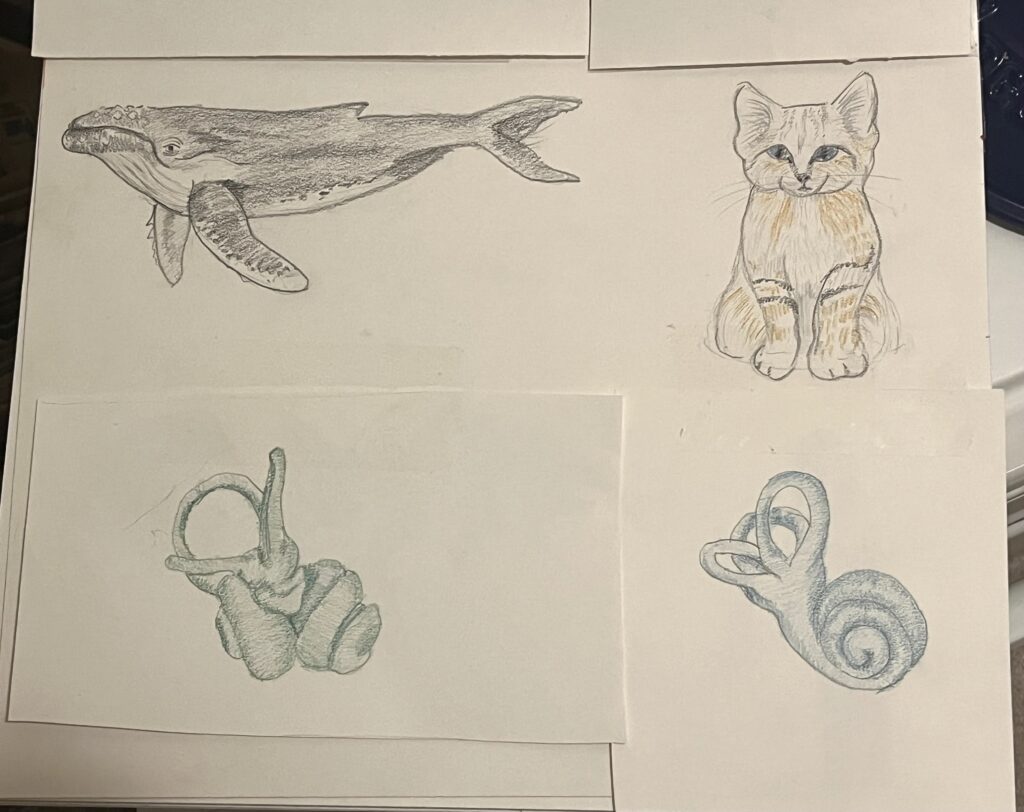
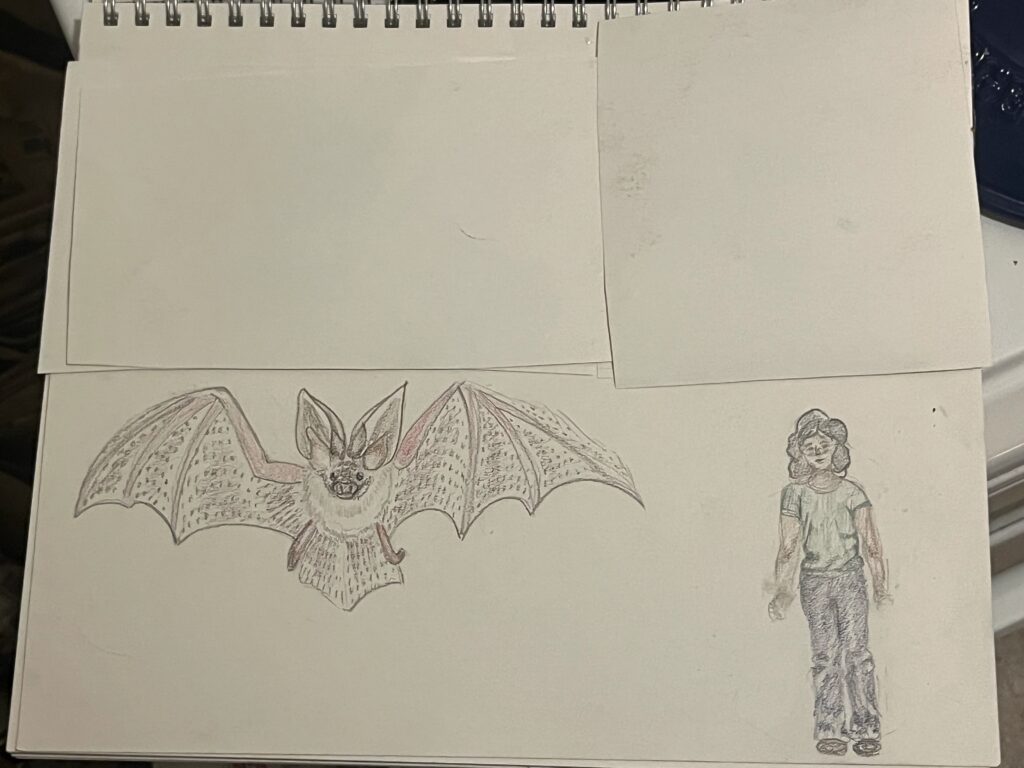
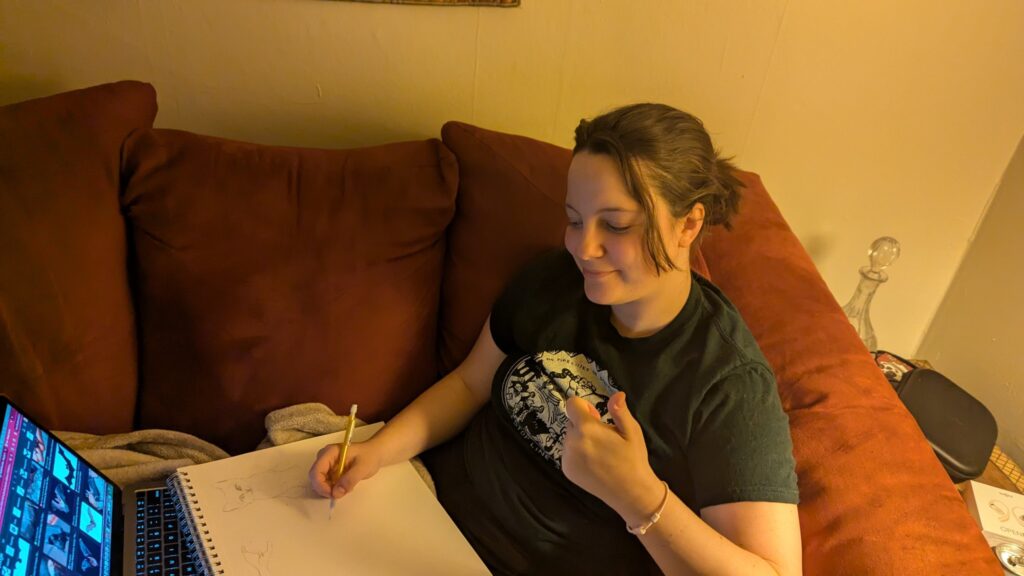
Key: First image: mysticete whale. Second image: Sand Cat. Third image: Long Snouted Bat. Fourth Image: Human!
Sensory acquisition is an organism’s method of interpreting its surroundings, so that it can effectively process and perform a context dependent response. For humans, one of our primary methods of environmental understanding is audition. The external human ear funnels pressure (sound) waves to the tympanic membrane, which vibrates middle ear’s ossicular bones in accordance to the sound wave’s frequency. The ossicular bones move the cochlear oval window, which produces correlatory waves within the cochlear fluid that moves the basilar membrane within the organ of corti at a location specific to the frequency being received. The hair cell mechanoreceptors bound to the corti move with the fluid and brush their microvilli against the tectorial membrane, inducing action potentials that indicate the cochlear location of activation, and inadvertently the sound frequency, to the brain. The structural and procedural components of human audition are heavily tailored to the needs, environment, and social behavior that humans participate in. However, other animal species live in significantly different conditions, which leads to large variability in auditory systems between animal species, including within a single genus, based on unique acoustic requirements. I will discuss the ear structures of whales, bats, sand cats, birds, and reptiles to highlight the importance of environmental properties, sensory integration with other senses, predator and prey dynamics, and social conditions on hearing capabilities and structural dynamism.
Environmental factors, such as what substance and climate the sound waves are traveling through, highly influence ear structure. Water attenuates light conduction much quicker than sound, which leads animals like whales to be more reliant on auditory transmission for environmental understanding than vision. Whales are one of the few mammals that have adapted an air-based ear structure to underwater sound transmission, and they perform the greatest range of sound frequencies out of the mammal class (peak at 200 khz and lowest at 0.05khz). For the whale’s external ear, they do not possess an auricle, and they possess a highly narrow ear canal. The middle ear is surrounded by a massive shell-shaped periotic bones called the tympanic bulla, and the inner ear is encapsulated by the periodic bula within a spongy mucosa cavity. These structures position the ear structure in line with the mandibular fat channels and pan bone. This positioning is highly important, for rather than sound predominantly entering through the ear canal entrance, sound conducts directly through the head due to similar impedances (a measure of opposition to electrical flow) between tissue and sea water. The sound travels through the lower jaw’s mandibular channel to the middle and inner ear. Similar to the Rine and Weber test, sound travels through the bone and fat to directly interact with the internal ear structure, rather than traveling through the ear canal. This method of sound conductance is highly specific to the salt water environments that whales live within. Whales have also adapted to auditory reliance by possessing cochlear ducts that are hypercellularized and overdeveloped. Cochlear pillar cells surrounding the hair cells are highly thick and dense within the lower basal turn (first turn of the cochlea). Additionally, whales have a proportionally higher density of auditory nerve fiber counts in comparison to ocular and vestibular counts. The cochlear structure and fiber count indicates the comparatively high development and utilization of the acoustic system. Another important quality of water is its density. Whale ears have to be designed to handle high degrees of pressure, especially for species that dive deep to hunt for food. Whales combat this conundrum by having middle ears that have thick eustachian tube walls that help prevent pressure induced tube closure, as well as extremely reduced vestibular system semicircular canals to prevent motion and altitude sickness. Whale sound usage is also influenced by social dynamics; whales that are solitary have a peak sound spectra of 100 kHz while whales that live in large social groups have peak spectras below 80 kHz.
Another animal that has altered ear morphology to be better suited for its niche habitat is the sand cat. The sand cat lives within highly arid environments where low frequencies travel significantly farther than higher pitched sounds. Potential predators and prey emit low frequencies that sand cats are able to register and respond to because of their middle ear adaptations. Sand cats are more sensitive to frequencies below 2 kHz due to increased middle ear length. The larger distance and higher air concentration within this region is hypothesized to increase the interaural time of arrival of the sounds from the same source, leading to higher sound specificity and locational accuracy. Similar adaptations have been seen in kangaroo rats who critically rely on acute hearing to avoid predators, for arid environments have low availability of vegetation to hide.
Bats occupy a temporal niche as nocturnal hunters. Bats have poor eyesight, and their insectoid prey are relatively small, so they rely on echolocation to pinpoint prey locations. Bats ears are highly attuned to receive and auditory stimuli and locate moving targets. Bats have one of the greatest vocalization frequency ranges of <20kHz to over 200 kHz, and as such, they must have a complex auditory system to process this information to target prey. Laryngeal echolocating bats have one of the best auditory systems for ultrasonic sound. Laryngeal echolocating bats have large cochleas with 2.5-3.5 turns with high structural variability between bat species based on their prey type and environment. For example, Percival’s Trident bat can hear frequencies at around 212 kHz while other members of its family hear up to 64-157 kHz. Percival Trident’s bat has a modified cochlear basal turn, higher pitch tonotopic organization, and decreased basilar membrane stiffness. In general, increased cochlear coiling and length in relation to body size was important for ultrasonic hearing required for nocturnal bug hunting.
Environmental, predatorial, food acquisitional, and social stimuli are highly variable, so the sensory organs devoted to understanding these stimuli must be adaptable for survival. These animal examples provide a small view into the ways in which auditory systems can evolve to match survival needs.
Literature Cited:
Davies, K. T., Maryanto, I., & Rossiter, S. J. (2013). Evolutionary origins of ultrasonic hearing and laryngeal echolocation in bats inferred from morphological analyses of the inner ear. Frontiers in Zoology, 10(1), 2. https://doi.org/10.1186/1742-9994-10-2
G., H., J., R., M., R., & W., P. (2002). Mammalian ear specializations in arid habitats: Structural and functional evidence from sand cat ( Felis margarita ). Journal of Comparative Physiology A: Sensory, Neural, and Behavioral Physiology, 188(9), 663–681. https://doi.org/10.1007/s00359-002-0332-8
Girdlestone, C. D., Piscitelli-Doshkov, M. A., Ostertag, S. K., Morell, M., & Shadwick, R. E. (2017). Description of Cochlear Morphology and Hair Cell Variation in the Beluga Whale. Arctic Science, AS-2017-0031. https://doi.org/10.1139/AS-2017-0031
Ketten, D. R. (1997). STRUCTURE AND FUNCTION IN WHALE EARS. Bioacoustics, 8(1–2), 103–135. https://doi.org/10.1080/09524622.1997.9753356
Negandhi, J., Bergevin, C., & Harrison, R. V. (n.d.). SCANNING ELECTRON MICROSCOPY OF THE BASILAR PAPILLA OF THE LIZARD (ANOLIS CAROLINENSIS).
Tucker, A. S. (2017). Major evolutionary transitions and innovations: The tympanic middle ear. Philosophical Transactions of the Royal Society B: Biological Sciences, 372(1713), 20150483. https://doi.org/10.1098/rstb.2015.0483